- Press Office
- Press releases 2000
- Discovery of a unique symbiosis betwee
Discovery of a unique symbiosis between bacteria and a marine worm (2001)
Dr. Nicole Dubilier, a scientist in the "Molecular Ecology" research group of Dr. Rudolf Amann at the Max Planck Institute of Marine Microbiology in Bremen, and her colleagues have discovered a unique symbiosis between bacteria and a marine worm described recently in the journal Nature (Dubilier, N, Mülders, C, Ferdelman, T, de Beer, D, Pernthaler, A, Klein, M, Wagner, M, Erséus, C, Thiermann, F, Krieger, J, Giere, O, and Amann, R. 2001. Endosymbiotic sulfate-reducing and sulfide-oxidizing bacteria in an oligochaete worm. Nature 411:298-302.
Without symbioses, life on earth as we know it today would not exist. Symbioses between bacteria and primitive one celled organisms were crucial for the diversity and evolution of multicelled eukaryotic organisms. Every human cell contains the remnants of bacterial symbionts in the form of mitochondria, organelles without which we could not breathe. More recent evolutionary history also shows numerous examples of successful associations between bacteria and eukaryotes, and there is hardly a plant or animal group that does not profit from bacterial symbionts. The enormous diversity of bacterial symbioses is only slowly being discovered, in particular since the introduction of molecular methods to microbial ecology about 10 years ago. Since most bacterial symbionts remain as yet unculturable, little was previously understood of their identity and function. Using molecular and biogeochemical methods, scientists from the Max Planck Institute of Marine Microbiology in Bremen and their colleagues have discovered a unique symbiosis between bacteria and a marine worm, a further example for the remarkable diversity of bacterial symbioses.
The term symbiosis typically evokes an image of beneficial interactions between two individuals, the symbiont and the host. Associations with multiple endosymbionts are assumed to be rare because competition between symbionts could be harmful to the host, suggesting that in most symbioses three is a crowd. Dr. Nicole Dubilier and her colleagues present evidence for a symbiosis in which two symbionts not only share a mutualistic relationship with their host but also with each other. This unusual "ménage à trois" occurs in a gutless marine worm from the animal group Oligochaeta.
Dr. Dubilier has been collaborating for many years with Dr. Olav Giere (University of Hamburg) and Dr. Christer Erséus (Swedish Museum of Natural History) on studies of bacterial symbioses in gutless oligochaete worms. These marine relatives of earthworms have no mouth or gut and are dependent on their symbionts for their nutrition. All gutless oligochaetes harbor sulfide-oxidizing bacteria as their primary symbionts, and were previously only known to occur in marine sediments in which sulfide is present. The main source of sulfide in marine sediments is sulfate-reducing bacteria. These bacteria use organic carbon compounds as an energy source and sulfate as an oxidant or electron acceptor, which is reduced to sulfide during a process called dissimilatory sulfate reduction.
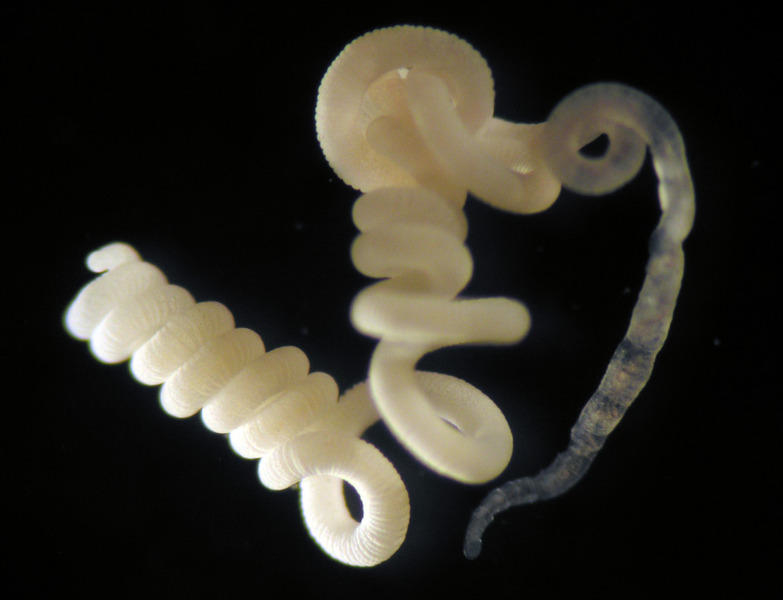
One of the essential requirements for chemoautotrophic symbioses is the availability of reduced sulfur compounds, such as sulfide. Without this energy source, the bacteria would not be able to feed their hosts, and these would eventually starve. Dr. Dubilier and her colleagues were therefore very surprised when they discovered an oligochaete worm with chemoautotrophic symbionts thriving in an environment in which, at first, they were not able to detect any sulfide. The worm, with the Latin name of Olavius algarvensis, was discovered in a small Mediterranean bay off the island of Elba (Italy) (Fig. 1 and 2). The worms live at 8 - 10 meters water depth near sea grass beds in coarse sediments 5 - 15 cm below the sediment surface. Directly under the skin of these worms the scientists observed, next to the already known primary sulfide-oxidizing symbionts, unique sulfide producing bacteria as secondary symbionts. This discovery immediately gave the scientists a clue as to how the symbiosis could survive without sulfide in the environment: the secondary symbionts produce sulfide, which is used by the primary symbionts as an energy source for the fixation of CO2. Using a wide array of modern molecular and biogeochemical methods, Dr. Dubilier was able to confirm this hypothesis in an intensive interdisciplinary collaboration with colleagues at the Max Planck Institute of Marine Microbiology and other institutes.
and her colleagues found the gutless worms at a water depth
of 8 meters.
a fluorescence microscope. The symbionts are
labled with specific gene probes in different colors.
The red shows the sulfate reducer, the green the s
ulfide oxidizer. The size bar corresponds to
0,02 millimeters. (Copyright NATURE)
But were the sulfate reducers active in the worm? Did they produce sulfide in their host? To answer this question Dr. Dubilier and two of her colleagues, Dr. Dirk de Beer and Dr. Tim Ferdelman from the Max Planck Institute in Bremen, developed a clever experiment. The scientists incubated the worms in radioactive sulfate and inserted an extremely thin silver needle into the worms. When they exposed the needles to an x-ray film after the experiment, a black signal appeared. This blackening occurred because the bacteria reduced the radioactive sulfate to sulfide, which precipitated on the needles. The experiment only worked under low oxygen concentrations. At higher oxygen concentrations, the symbionts no longer reduced sulfate to sulfide, a behavior common to almost all free-living, non-symbiotic sulfate reducers. These results were crucial in proving that the sulfate-reducing symbionts are active inside the worm and able to produce sulfide.
A final unresolved question remained: do the sulfide-oxidizing symbionts really need the sulfide produced by the sulfate-reducing symbionts? In their first experiments, the scientists had not been able to measure sulfide in the wormís environment, but they were worried that the methods they used might not be sensitive enough. They knew that free-living sulfide-oxidizing bacteria can gain energy even at very low sulfide concentrations. It was therefore possible that the sulfide oxidizers did not need the sulfide produced by the sulfate-reducers. To answer this question, the scientists developed a more sensitive method for measuring sulfide in the wormís environment. They discovered that the sediments contained only minute amounts of sulfide, comparable to concentrations in open sea waters, which are considered free of sulfide. The comparison of sulfide flux from the sediment (that is, the amount of sulfide that diffuses into the worm within a defined time period) with the internal production of sulfide from the symbionts showed that internally produced sulfide generally exceeded sulfide flux from the environment by at least 7 times, and sometimes by as much as 30 times. These results clearly demonstrated that the sulfate reducers are the main source of sulfide in this system.
How does this symbiosis work (Figure 4)? The sulfate-reducing symbionts produce sulfide, which is taken up by the neighbouring sulfide-oxidizing symbionts and converted to oxidized sulfur compounds such as sulfate. These oxidized sulfur compounds are in turn taken up by the sulfate-reducing symbionts and converted to reduced sulfur compounds such as sulfide. At first glance this cyclic or syntrophic sulfur cycle may seem to be a "Perpetuum mobile". However, for the worm and its symbionts to grow, energy sources must be taken up from the environment, such as dissolved organic compounds that occur in the pore waters of marine sediments.
What does the worm gain from this arrangement? The cycling of metabolic products between the two symbionts increases their energy yield, which in turn would be passed on to their host. Another advantage for the worm is that the sulfate reducers could take up metabolic byproducts that would otherwise be excreted. These waste products, called anaerobic metabolites (for example, succinate), are produced by marine animals as soon as environmental oxygen concentrations become limiting. Instead of excreting these waste products, the worms can reuse them in a kind of internal "symbiotic recycling" and thereby save additional energy. There is a further advantage to having a symbiotic sulfate reducer: these supply the worms and their primary sulfide-oxdizing symbionts with a continuous source of internal
Please direct your queries to:
Director
MPI for Marine Microbiology
Celsiusstr. 1
D-28359 Bremen
Germany
Room: |
3241 |
Phone: |
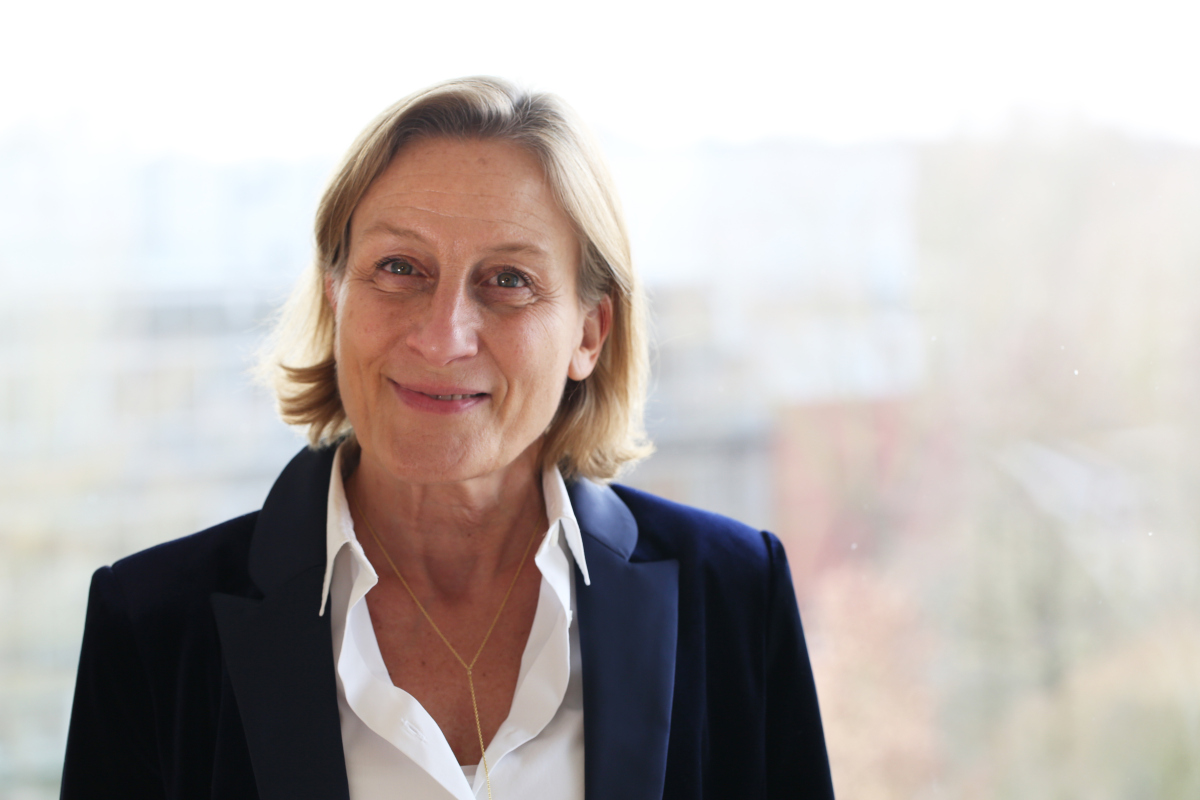
Head of Press & Communications
MPI for Marine Microbiology
Celsiusstr. 1
D-28359 Bremen
Germany
Room: |
1345 |
Phone: |
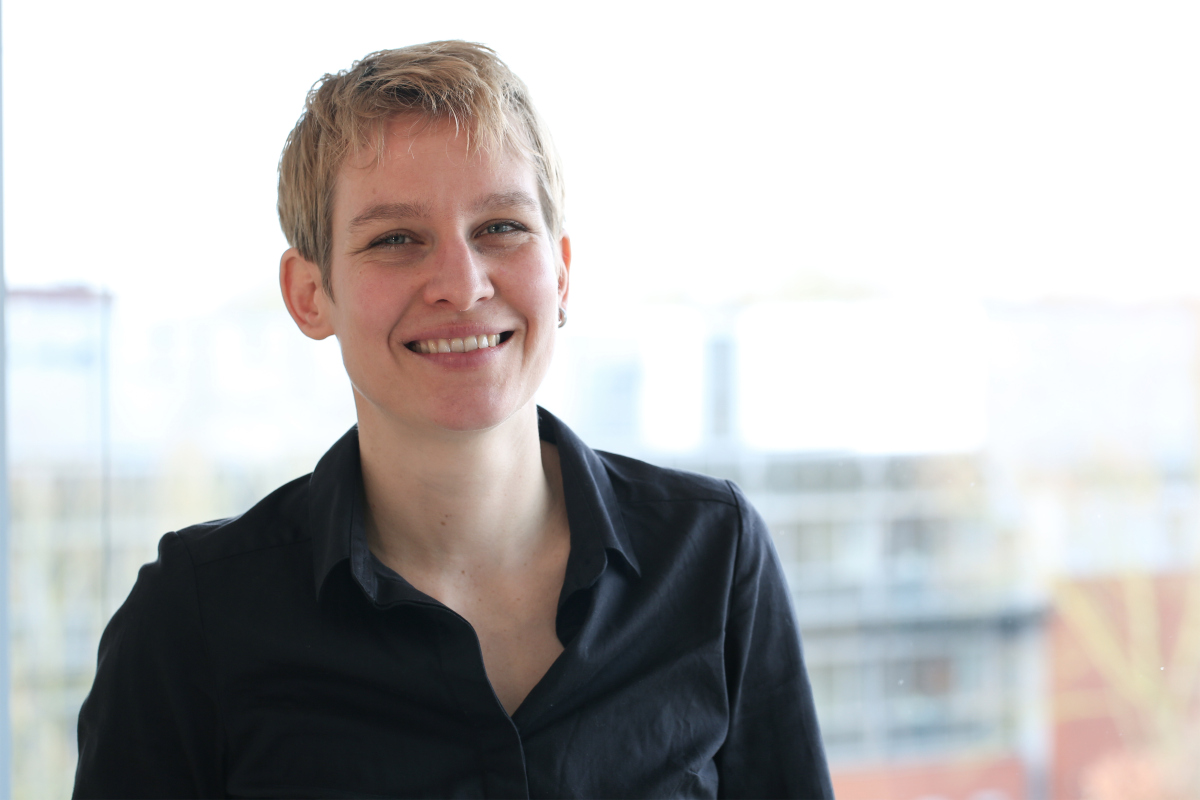